Electricity
The electric sector plays a central role in achieving economy-wide net-zero targets. In all scenarios, electricity demand increases over time as a result of direct electrification at the end-use and, in some cases, indirect electrification via electrolytic hydrogen production. At the same time, the carbon intensity of the generation mix declines over time in all scenarios, with nearly all generation coming from low- or zero-carbon sources in the net-zero scenarios. Because of the relatively low cost of renewable energy and opportunities for carbon capture and nuclear power, combined with the increasing competitiveness of electrification at the end-use, building a decarbonized electric grid is a core strategy for achieving economy-wide net-zero targets.
Figure 21 shows electricity supply and demand across scenarios compared with today. The most significant driver of increased load is the electrification of transportation, which results in around 1,800 TWh of new load by 2050 in the reference scenario, resulting in total demand roughly 30% higher than current today. Although there is also some electrification in buildings and industry, particularly increased adoption of heat pumps for space and water heating, this new load is offset by efficiency, resulting in relatively flat demand from these sectors. In the Net-Zero scenarios, there is additional direct electrification in the buildings and industry sectors, resulting in 40–60% higher end-use load relative to today. End-use electricity demand is higher in Higher Fuel Cost and Limited Options net-zero cases due to strengthened incentives for electrification via higher relative non-electric fuel prices. Electricity can also be used indirectly as an input to hydrogen production from electrolysis, synthetic fuel production, or direct air capture. In the Net-Zero Limited Options scenario, the indirect use of electricity for electrolysis becomes very large, accounting for around 4000 TWh in 2050, similar in scale to total generation today. In this case, electricity for hydrogen production represents 40% of total electricity demand, leading to a total increase in the scale of electric generation of 250% relative to today.
Electric generation in the Reference scenario shifts to primarily natural gas with the retirement of existing coal and nuclear, while renewables increase partly due to declining costs and partly to state-level incentives. In the Net-Zero All Options scenario, wind and solar expand more rapidly, providing nearly 50% of generation, with most of the rest coming from new gas with CCS and existing nuclear and hydro. There is a small amount of bioenergy with CCS in power generation, which produces negative emissions, although the majority of bioenergy with CCS is deployed in the liquid fuels sector. Gas without capture contributes a small percentage of generation, but it plays an important role as a firm capacity balancing renewables. In the Net-Zero Higher Fuel Cost scenario, gas with CCS is more expensive, resulting in a larger share of wind and solar, combined with increased total generation in absolute terms due to additional electrification. In the Net-Zero Limited Options scenario, wind and solar are much larger in absolute terms, partly because gas with CCS is unavailable but also because of the increased demand for electricity for hydrogen production. There is also an expansion of nuclear as a complementary source of zero-carbon power, with firm balancing provided by RNG, hydrogen, and battery storage.
The installed capacity mix for electricity supply is shown in Figure 22, including storage resources. This mix is distinct from the generation mix because different resources operate at different capacity factors. In particular, firm or dispatchable capacity is needed to balance renewables, but may only be needed infrequently. Thus there remains a large role for conventional gas-fired capacity in all net-zero scenarios. In all scenarios, new conventional gas capacity is part of the least-cost resource mix to achieve the 2050 target. This result is important from the perspective of the near-term emissions reduction strategy to replace retiring coal capacity with a combination of new gas and renewables. In the Net-Zero Higher Fuel Cost scenario, there is less deployment of gas with CCS but more conventional gas capacity to balance higher renewable penetration. In the Net-Zero Limited Options scenario, the gas mix shifts primarily to renewable and synthetic natural gas, and gas capacity is augmented by hydrogen-fired capacity, with small amounts of hydrogen produced from electrolysis being used for generation in peak hours as a form of long-duration storage. Battery storage also contributes to firm capacity in all scenarios.[1] In this scenario, there is also around 60 GW of new nuclear deployment. In all scenarios—especially those with very large increases in wind and solar—there is a significant expansion of inter-regional transmission capacity to facilitate regional balancing and allow net exports of power from higher-quality renewable resource areas, as shown in Figure 23.
There are significant differences in the projected energy and capacity mix across regions. Figure 24 shows regional totals for the East, South, Midwest, and West for the Net-Zero All Options and Limited Options scenarios. Deployment of wind and solar is concentrated in regions with greater resource availability and quality—in particular, wind in the Midwest and solar in the West. Regions with lower renewable potential, in particular the South, have the greatest deployment of gas with CCS and new nuclear in the Limited Options scenario and rely to a greater extent on net imports (reflected in Figure 24 by the gap between the top of the generation bars and the marker for the total plus electrolysis demand). In the Net-Zero Limited Options scenario, electricity demand for electrolysis is also primarily located in the Midwest and West. From a capacity perspective, all regions maintain sufficient firm resources to balance intermittent energy and ensure the adequacy of supply. Note that demand for electrolysis is assumed to operate flexibly and dispatch predominantly during periods of high renewable output (see Figure 25) and thus does not drive additional firm capacity needs.
The average wholesale price of electric generation (including both capacity investment and dispatch costs is higher in the Net-Zero scenarios than in the Reference scenario, reflecting the incremental cost of shifting to a low-carbon generation mix (see Figure 21). In the Reference scenario, a low gas price combined with falling costs of wind and solar lead to a reduction in the national average generation price relative to today of around $11/MWh, or roughly 1 cent/kWh, in real terms (expressed in 2015 dollars). In the Net-Zero All Options scenario, the cost of carbon capture and higher shares of intermittent renewables (leading to lower utilization of balancing resources) results in a generation price around $18/MWh higher than the Reference scenario in 2050, which is only $7/MWh higher than current prices in real terms. In the Net-Zero Higher Fuel Cost scenario with higher costs for natural gas, biomass, CO2 transport and storage, and implicitly higher carbon prices, the price of electricity rises to $82/MWh, or $31/MWh (roughly 3 cents per kWh) higher than the Reference scenario. In the Net-Zero Limited Options scenario, the average generation price increases to $95/MWh, reflecting a more expensive mix of new nuclear, very higher renewable shares, and balancing capacity from storage, hydrogen, and gas.
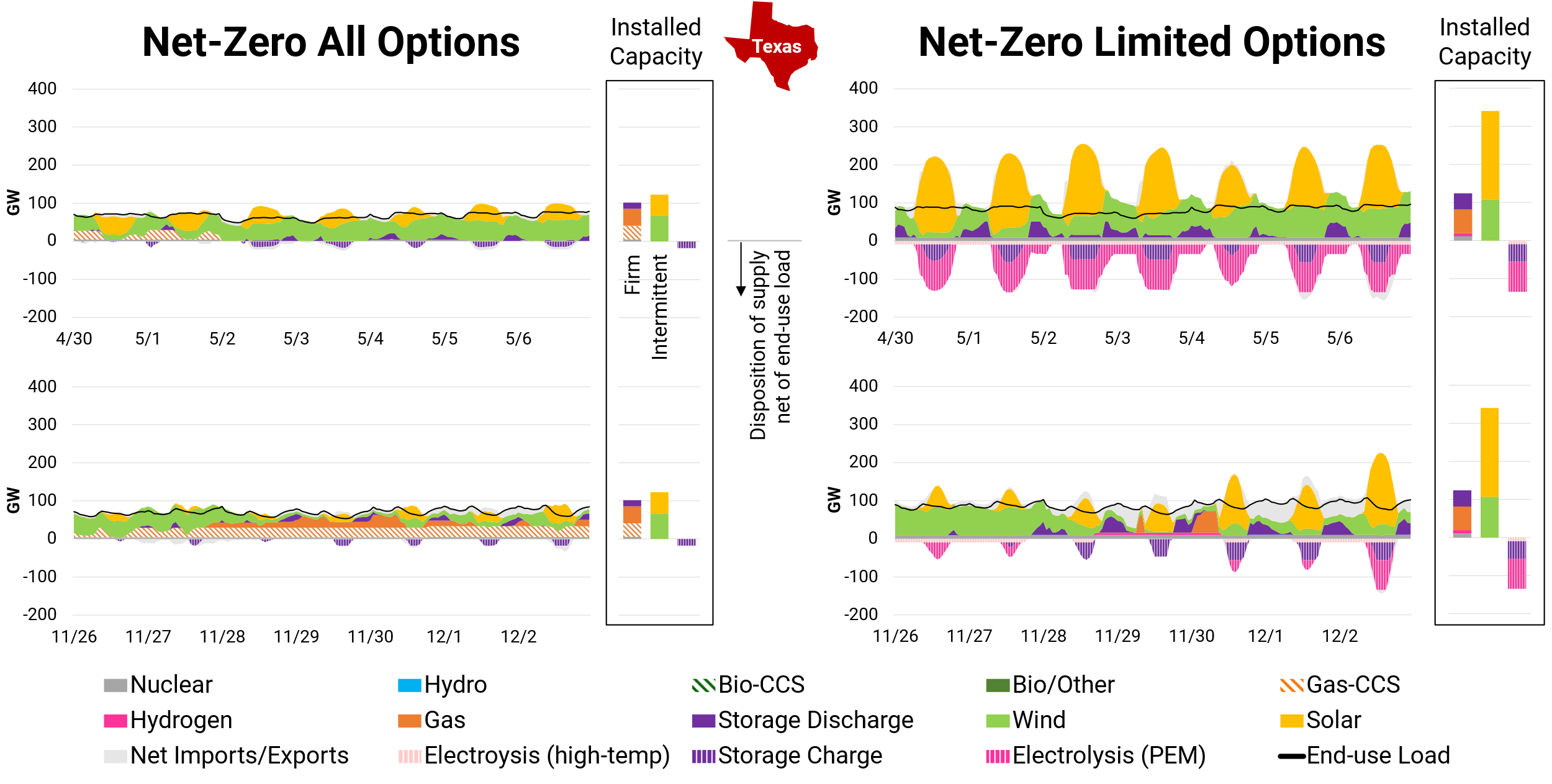
To illustrate the impact of these changes to the electricity supply mix in the net-zero scenarios, Figure 25 shows an example of hourly dispatch and disposition of load by resource type in the Texas model region in 2050. The two sample weeks shown reflect two ends of the spectrum: one in which renewable output is high relative to end-use load (May) and one with low renewable output and relatively high end-use load (November). Other weeks in the year generally fall between these two extremes. In the Net-Zero All Options case, wind and solar provide most of the energy in the May week, with storage and trade with neighboring regions providing balancing (note that new transmission has increased the interconnection capacity between Texas and the East and West). All existing nuclear capacity remains online with license extensions. Nuclear operates most of the year but is assumed to have flexibility to turn down during periods of high renewable output. Gas with CCS is deployed sparingly in the May week, dispatching flexibly during night hours at the beginning of the week and not at all later in the week. Whether gas with CCS technologies can operate this flexibly is an important question for future research. In the November week, wind resources fall to near zero during the middle of the week, which—combined with reduced solar output and high space heating load from colder temperatures—drives the need for firm dispatchable resources. Gas with CCS runs at full capacity for most of the week, and conventional gas is dispatched during the tightest period in the middle of the week. This November week is one of the only instances during the modeled year where conventional gas is dispatched; thus, it has a very low annual capacity factor. In contrast, gas with CCS generally operates more frequently in baseload mode. In the Texas region, its average annual capacity factor is around 50%, which is lower than the national average of around 63% for gas with CCS, reflecting the higher shares of renewables in Texas.
In the Net-Zero Limited Options scenario, there is much higher installed capacity of wind and solar, driven by increased demand for electricity for hydrogen production and the constraint on CCS deployment. In this scenario, flexible dispatch of electrolysis is timed to coincide with solar output. In the May week with high output from wind and solar, battery storage is used to increase the capacity factor of electrolysis by allowing hydrogen production to continue after dark. As discussed in the section on Hydrogen and Hydrogen-Derived Fuels, there is also hydrogen production from a high-temperature electrolysis technology that uses thermal input from nuclear to operate more efficiently. These two resources operate together at a nearly constant capacity factor during the May week, but electrolysis dispatch is more limited in the November week when supply conditions are tight. In fact, these two weeks correspond to the maximal and minimal weeks for hydrogen production during the modeled year. In the November week, gas and hydrogen capacity are used for brief periods to balance supply during the renewable drought. Imports from neighboring regions are also used (as available) depending on market conditions in neighboring regions, but the model requires that sufficient firm capacity be available within the model region. Two key observations are consistent across both scenarios: firm gas and/or hydrogen capacity is needed for resource adequacy but will operate infrequently, and many hours of the year experience high shares of dispatch from inverter-based resources, creating operational challenges for grid stability.
Changes in end-use demand (discussed further in the Energy End-use section) also have implications for the electricity system. Figure 26 shows the annual hourly load shape for two model regions, New York and Southeast, in both the base weather year of 2015 and the Net-Zero Limited Options scenario in 2050. The increased electrification of space heating and vehicle charging, which requires more energy in colder temperatures, leads to a structural shift toward higher winter peaks. Meanwhile, efficiency improvements in cooling and other end-uses offset load growth during the summer and other parts of the year. In colder climates, electrification for space heating increases but—in many cases—with a hybrid configuration of a heat pump with gas backup, which avoids steeper increases in peak electricity demand during the coldest hours. In warmer climates, all-electric heat pumps are more cost-effective and provide a larger share of peak heating demand. Efficiency improvements in heat pumps also help to limit the increase in winter peak load. Vehicle charging shapes are based on an assumed mix of different behavior patterns, including end-of-day and overnight charging by residential consumers, as well as daytime charging by fleets and at workplaces and other commercial locations. This mixture leads to a more even distribution of diurnal load than would occur with more pessimistic assumptions about uncoordinated charging concentrated into shorter periods, illustrating the importance of managing charging load shapes. Future research will further explore the value of flexible charging and other forms of demand-side management.
The electricity model also includes compressed air energy storage (CAES), and a generalized bulk storage technology representing a range of emerging technologies such as flow batteries, liquid air storage, or various forms of thermal storage. Future modeling studies will incorporate additional electric storage technologies in more detail. ↩︎